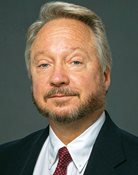
Fall 2020 - Vol. 15, No. 3
Defining Features of Severe Acute Respiratory Sydrome:
What Makes Coronavirus-2 (SARS CoV-2) Such a Formidable Pathogen
Joseph M. Kontra, M.D.
Division Chief, Infectious Diseases, Penn Medicine Lancaster General Health
“Those who do not learn from history are doomed to repeat it.”
—George Santayana (1863-1952)
INTRODUCTION
In December of 2019 China identified an outbreak in Hubei Province of an acute respiratory infection caused by a novel coronavirus. This new disease, designated by the World Health Organization (WHO) as COVID-19, would rapidly evolve into the worst global pandemic since the 1918 Spanish Influenza. As of July 27, 2020, worldwide it had resulted in 16,264,048 confirmed infections and 648,966 deaths,1 and it remains uncontrolled globally, especially in the United States.
There are four endemic strains of human coronavirus (229E, NL63, OC43, and HKU1) that typically cause mild and self-limited winter seasonal upper respiratory infections. There have also been three human epidemics caused by zoonotic transmission of coronaviruses endemic in bats, with subsequent sustained human-to-human transmission. These include Severe Acute Respiratory Syndrome (SARS) in 2002-2003, and Middle Easter Respiratory Syndrome (MERS) in 2014. The third zoonotic pandemic, SARS CoV-2, has proven to be far more contagious than either SARS CoV or MERS. COVID-19 demonstrates a complex pathophysiology that leads to varied and perplexing clinical presentations, with unique transmission characteristics that defy standard measures of infection control.
This paper will explore the unique characteristics of SARS CoV-2 that have made COVID-19 such a profoundly formidable pathogen, and will draw comparisons and lessons learned from the 1918-19 Spanish Influenza pandemic.
VIROLOGY
SARS CoV-2 is closely related phylogenetically to another beta-coronavirus, SARS CoV, that caused a pandemic in 2002-2003. Both enter human cells via attachment to the angiotensin-converting enzyme (ACE) receptor by virtue of very similar surface spike binding proteins. While ACE2 receptors are expressed in a wide variety of human tissues including throughout the GI tract, they are highly expressed in human nasal mucosal cells, along with an entry-facilitating transmembrane protease.2 Expression of ACE2 proceeds along the entire respiratory tract in a decreasing gradient from nose to lungs, and is the primary mechanism of acquisition of COVID-19.3
Strain variation within SARS CoV-2 has been demonstrated, and it is interesting to note that the L-type strain that was epidemic in China is now less common than the S-type strain currently circulating outside of Asia.4 In addition, since March 2020 an additional strain mutation has dominated the landscape, called D614G (aspartic acid is changed to glycine at site 614), or simply the “G-strain.” This mutation affects three key loci in the complex conformational structure of the spike binding protein, resulting in more avid binding to the human ACE2 receptor, with facilitated and more efficient entry into the cell. In addition, the mutation seems to be associated with higher viral loads in infected patients.5 Interestingly, the mutation is not associated with an increase in disease severity, although it clearly provides a fitness advantage by increasing infectivity.
This is a common theme in viral evolution: increased efficiency of reproduction and transmission, without added harm to the host. In Arizona, the U.S. state with the most explosive epidemic of COVID-19 at the time of this writing, the D614G mutation is present in 90% of viral isolates that have undergone genomic analysis.
MECHANISMS OF TRANSMISSION OF SARS COV-2
The predominant mode of spread of SARS CoV-2 is direct person-to-person transmission via respiratory droplets. Secretions from infected COVID-19 patients may contain 105-107 viral copies per ml,6 and droplets are produced and easily disseminated not only by coughing or sneezing, but also by shouting, singing, or talking.7 Dispersal of such droplets is generally limited to a radius of about 6 feet over several minutes. Subsequent environmental contamination with SARS CoV-2 RNA has been well documented,8 making spread by fomites possible by self-inoculation of mucous membranes.
True airborne spread of COVID-19, like measles, varicella, or tuberculosis, has been postulated based on production of suspended micro-droplets during coughing, and the detection of SARS CoV-2 fragments of viral RNA in those particles.9 Epidemiologic transmission studies, however, have not found clear evidence that true airborne spread of COVID-19 is a significant mechanism of contagion, outside of specific aerosol-generating medical procedures.
Neither the Centers for Disease Control and Prevention (CDC) nor the World Health Organization (WHO) has previously accepted the possibility of airborne transmission of SARS CoV-2, but based on the aforementioned in vitro and artificial laboratory experiments, a group of 239 scientists petitioned the WHO to re-examine that mechanism.10 WHO subsequently published a scientific brief recognizing the emerging evidence of possible airborne transmission, but correctly concluded that much more study is needed, and made no additional recommendations regarding infection control protocols.11 It is clear from studies in patients that the detection of viral RNA fragments in aerosols does not mean that such micro-particles are infectious.12 Epidemiologic studies during outbreaks also do not support true airborne spread of COVID-19.13
SARS CoV-2 RNA has been detected by PCR (polymerase chain reaction) in multiple specimen types from outside the respiratory tract, including blood, ocular fluids, semen, and stool. Cultivation of infectious virus from these routes, however, has only been rarely documented (stool), and none of these routes is felt to be a significant source of contagion of COVID-19.
ASYMPTOMATIC AND PRE-SYMPTOMATIC TRANSMISSION
Asymptomatic transmission of SARS CoV-2 is one of its most defining features, as asymptomatic infections have been estimated to account for up to 30%-40% of all COVID-19 infections.14 Such transmission can occur for approximately one week. Pre-symptomatic transmission of virus can occur an estimated 2-3 days prior to the onset of illness.15 This has proven to be an essential feature of SARS CoV-2, and one that led to an inability to control the virus with traditional public health measures. In contrast, transmission of influenza occurs 1 day prior to illness onset. And for SARS CoV (2003), virus transmission begins with the onset of symptoms, a feature that facilitated eventual control of that pandemic through prompt isolation of symptomatic patients.
SARS CoV-2 viral RNA can be detected by PCR for 6 weeks or longer in recovered patients, depending on the severity of illness. However, it is important to recognize that detectable viral RNA does not indicate that live transmissible virus is present.16 Both viral cultivation studies and epidemiologic studies of secondary transmission suggest that infectivity beyond 8 days of illness in a clinically recovered patient is very rare.17.18 Additional support for the same infectivity period comes from separate contact tracing studies.19 These studies and others have prompted the CDC and health systems, including the University of Pennsylvania Health System, to move from test-based to time-based strategies for determining the duration of quarantine and isolation precautions in COVID-19 cases.
IMPLICATIONS FOR PREVENTION of INFECTION
The science of viral transmission of SARS CoV-2 informs the critically important policies for infection control using PPE (personal protective equipment) for health care personnel (HCP) interacting with COVID-infected as well as COVID-unknown patients. Proper donning of surgical masks (or respirators for aerosol-generating procedures), eye protection, gloves, and gowns is highly effective in reducing the risk of HCP infection with SARS CoV-2. In an extensive study from Wuhan, China, HCP wearing proper PPE neither tested positive by PCR for SARS CoV-2, nor seroconverted, during several consecutive months of surveillance.20
Breaches in PPE protocols, however, can and have resulted in HCP acquisition of COVID-19. In a broad serologic survey, 6.4% of HCP were found to have IgG to SARS CoV-2 nucleocapsid protein, although household exposures were recognized as a possible factor.21 In a United Kingdom teaching hospital where standard PPE included only scrubs and surgical masks, 3% of HCP tested positive for COVID-19 by nasal PCR, and a cluster of genetically related virus in a non-COVID ward suggested possible HCP-to-HCP transmission.22 It is now recognized that it is critical to cover all exposed mucous membranes with a face mask and eye protection for all HCP-patient interactions. For aerosol-generating-procedures, an N95 respirator or Powered Air-Purifying Respirator (PAPR) is required.
In a meta-analysis of 172 observational studies of the effects of masking, eye protection, and physical distancing on transmission of COVID-19, SARS, and MERS, all three interventions were shown to provide substantial reductions in the risk of viral transmission.23 Face masks reduced the risk of transmission by 85%, eye protection with goggles or face shield by 78%, and social distancing of at least 1 meter by 82% (2 meters, or 6 feet, is recommended by CDC). For N95 respirators the risk reduction was 96%.
Combinations of interventions were not studied. Nonetheless the message is clear. Individuals are capable of adopting these simple self-protective behaviors of social distancing and wearing a face mask, and in so doing protect not only themselves but also those with whom they come into contact. This along with hand washing and other simple hygiene maneuvers minimizes the risk of acquisition of COVID-19.
HOST RESPONSE, PATHOPHYSIOLOGY, AND MULTISYSTEM CLINICAL DISEASE
In immune competent patients, infection of respiratory tract cells by SARS CoV-2 results in a prompt immune response invoking both humoral and cell-mediated immunity. This process is most often controlled by a balance of anti-inflammatory and pro-inflammatory physiologic responses.
About 80% of COVID-19 cases are mild and do not require hospitalization, with only 15% manifesting as more severe disease requiring admission, and 5% requiring intensive care.24 Typical symptoms include cough, fever, malaise, myalgia, sore throat, and a loss of taste and smell. Dyspnea may develop and be associated with findings of hypoxemia and ground glass opacities on chest CT imaging. Most cases are self-limited and last 1-2 weeks.
In severe cases, however, the pathophysiologic progression is more draconian. Lymphopenia resulting from both direct killing of T-cells and impaired lymphopoesis becomes profound. Subsequent breakdown of the respiratory epithelial-endothelial barrier occurs, accelerating the inflammatory response by the influx of neutrophils and macrophages. The resulting endotheliitis leads to pulmonary alveolar edema and hyalin membrane formation.25 Oxygen diffusion capacity rapidly declines. A cytokine storm ensues, characterized by elevated levels of C-reactive protein, D-dimer, ferritin, and Interleukin-6 (IL-6). Radiographic and clinical correlates include progressive ground glass opacities on CT imaging, increasing levels of hypoxia, and the development of Adult Respiratory Distress Syndrome (ARDS).26 Interstitial fibrosis develops as the inflammatory process continues.
As severe disease progresses, microthrombi develop as a consumptive coagulopathy begins. Outside of the lungs this manifests as thrombotic complications, including deep venous thrombosis, pulmonary embolism, ischemic stroke, limb ischemia, and myocardial infarction.27 Dysregulated inflammation progresses unrelentingly, resulting in viral-mediated septic shock with multi-organ failure, for which death becomes the almost inevitable outcome.28
The pathophysiology of COVID-19 extends far beyond the respiratory tract. In addition to the thrombotic complications outlined above, direct pathologic involvement of the GI tract, liver, renal, cardiovascular, neurologic, cutaneous, ocular, and reproductive systems has been described.29 These are outlined in Table 1.30
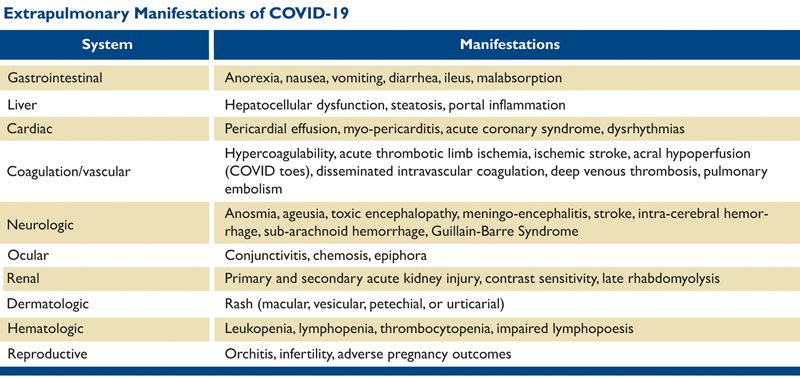
Table 1. From: Aboubaker A, Raba AA, and Aboubaker A. Extrapulmonary and atypical clinical presentations of COVID-19. J Med Virology June 10, 2020. doi: 10.1002/jmv.026157 and Behzad S, Aghaghazvini L, Radmard AR, et al. Extrapulmonary manifestations of COVID-19: Radiologic and clinical overview. Clin Imaging 2020 Oct; 66: 35-41. doi: 10.1016/j.clinimag.2020.05.013
Post-infectious syndromes have also been identified. Multisystem Inflammatory Syndrome in Children (MIS-C) is a post-infectious syndrome that resembles Kawasaki disease and toxic shock syndrome. Mucocutaneous, gastrointestinal, respiratory, and cardiovascular manifestations are common, with the onset about one month after a documented COVID-19 infection. In a review from New York, 80% required intensive care, and 10% mechanical ventilation.31 Guillain-Barre syndrome has also been described as a post-infectious sequelae of COVID-19.32
ABO blood group also seems to affect the outcome in COVID-19 infection. Blood type A has been associated with more severe infection with COVID-19, whereas blood group O is associated with less severe disease. A human nucleotide polymorphism at locus 9q34.2 has been associated with the propensity of blood type A for severe disease.33
IMPLICATIONS FOR CLINICAL MANAGEMENT
Successful management of many COVID-19 patients can be accomplished with supportive oxygen administration. High-flow O2 is now favored over early mechanical ventilation for those patients who deteriorate. Prone positioning is often beneficial with or without mechanical ventilation. Therapeutic interventions for COVID-19 are targeted either at the virus itself or the host immune response.
A National Institutes of Health (NIH) clinical trial of the RNA polymerase inhibitor remdesivir demonstrated a statistically significant reduction in time to recovery from 15 to 11 days, a 31% improvement.34 The same study also demonstrated reduced mortality from 11.6% to 8% in the remdesivir arm, although that fell just short of statistical significance (P = 0.059). Remdesivir (Gilead) is now available under an FDA emergency use authorization (EUA). No other antivirals have yet proven efficacious, and multiple trials of hydroxychloroquine have failed to show clinical benefit.
Treatment of COVID-19 patients with COVID convalescent plasma (CCP) is currently utilized under a number of FDA Investigational New Drug protocols. In theory, providing patients with exogenous anti-SARS CoV-2 antibodies during acute illness (prior to host antibody production) may enhance recovery. An early study35 did not indicate improvement in time to clinical improvement with CCP, but there was a mortality reduction from 24% to 15.7%. That difference was not statistically significant, but it should be noted that the study was terminated early due to lack of enrollment (waning of the epidemic in the study institutions) and so was under-powered. Large national protocols are active, and additional data will provide the ultimate answer. Combination monoclonal antibodies to SARS CoV-2 are also under study.
Attempts to modulate the pro-inflammatory immune response are yet another strategy to combat COVID-19. Dexamethasone, 6mg orally or IV daily has been shown in the RECOVERY trial to lower the incidence of death compared with usual care in patients receiving invasive mechanical ventilation (29.3% vs. 41.4%) or oxygen without invasive mechanical ventilation (23.3% vs. 26.2%), but not among those who were receiving no respiratory support at randomization (17.8% vs. 14.0%).36
An additional strategy targeting the inflammatory cytokine IL-6 is being studied in more than a dozen clinical trials.37 Tocilizumab is available and is being used as an off-label treatment for COVID-19 in patients with elevated inflammatory markers suggestive of cytokine storm. A recent study demonstrated that, compared to placebo, tocilizumab significantly reduced mortality from 20% to 7%, and reduced the risk of mechanical ventilation.38 Patients who received tocilizumab, however, did experience an increase in secondary infections.
As a result of the thrombotic tendencies associated with COVID-19, anticoagulation therapy is also now a standard of care for COVID-19 patients. Protocols are included in the CDC clinical guidance for COVID-19 management.39
DIAGNOSTIC TESTS FOR COVID-19
Polymerase Chain Reaction (PCR) is the primary laboratory test for the diagnosis of COVID-19. Many platforms are available, and most have an analytic sensitivity down to 20-100 copies/ml of respiratory secretions. Nasopharyngeal swabs and anterior nasal swabs are equivalent in terms of yield, although lower respiratory tract samples have a higher yield in intubated patients.40 Clinical sensitivity is less and is largely dependent on pre-analytic factors such as specimen quality, transport conditions, and laboratory procedural precision. In general, sensitivity of PCR is estimated to be 80%-90%, with specificity of 99.6%. More important is the predictive value of the test, which is dependent on the pre-test probability of infection, or the prevalence of COVID-19 in the population being tested. For example, with the above assumptions in a population with 5% prevalence, the positive predictive value (PPV) of PCR is 91%, and the negative predictive value (NPV) is 99%. In this scenario, repeating a negative test is unnecessary. Alternatively, for a population with 30% prevalence of disease (e.g. Arizona), the PPV is now 99%, and the NPV 95%. Here, repeating a negative test may be warranted.
By comparison, the precise nature and significance of the human antibody response to COVID-19 infection remains puzzling. The majority of immunocompetent patients will have developed a detectable antibody response by 14 days after the onset of illness. IgA is the first antibody to appear late in the first week of infection, followed a few days later by IgM. IgG antibodies arise during the second week of illness.41 Antibodies to both the SARS CoV-2 nucleocapsid and to the spike protein are characteristic. Neutralizing antibody, at least in vitro, is associated with antibodies to the spike protein antigen.
While many antibody tests are available through FDA Emergency Use Authorization, we have not yet determined which antibody assay best correlates with immunity. In addition, many assays have limited sensitivity and specificity performance characteristics. This combination of features precludes the use of COVID-19 antibody testing as an immunity card or a back-to-work card. Using SARS CoV-2 serology in the management of individual patients is not currently recommended. The development of an accurate serologic test for COVID-19 infection, however, will be key to evaluating the effectiveness of a future SARS CoV-2 vaccine.
Patients with asymptomatic COVID-19 infection have IgG antibody responses that are of lower magnitude and shorter duration than patients with symptomatic disease.42 About 80% of patients, with or without symptoms, had detectable IgG 3-4 weeks after exposure. Furthermore, 40% of asymptomatic patients became seronegative for COVID-19 IgG, vs. only 13% of symptomatic patients at follow up 8 weeks after hospital discharge. However, evidence demonstrating a robust cellular-mediated immune response to COVID-19 has now been demonstrated,43 and may provide protection against reinfection. Whether seronegative recovered COVID-19 patients are more susceptible to reinfection therefore remains unknown.
VACCINE DEVELOPMENT
There are more than 140 vaccine candidates in development, with about a dozen currently in at least phase II trials. The vaccine strategies include nucleic acid vaccines, recombinant proteins with adenovirus vectors, and live attenuated SARS CoV-2.44 The spike protein so important for binding to the ACE2 receptor seems an obvious target, as it may be the most likely to generate neutralizing antibodies.
In April 2020, the federal government initiated Operation Warp Speed to accelerate COVID-19 vaccine development. As of this writing, several vaccine candidates have reached advanced stages of scientific study.
Two nucleic vaccine candidates have gained ascendency. The first, a combined effort of the NIH and Moderna, targets the messenger RNA (mRNA) responsible for spike protein synthesis. It entered a phase III trial in mid-summer 2020 that is scheduled to involve 30,000 volunteers at 89 U.S. sites.
Another mRNA vaccine developed by Pfizer in combination with the German company BioNTech has demonstrated stimulation of both humoral and T-cell immunity, and also began phase III trials in mid-summer 2020. Unfortunately, mRNA vaccines suffer from a requirement for extremely low temperature storage, which may complicate attempts at global dispersion.
Several adenovirus vector vaccines have also reached late stage development. A simian adenovirus vector vaccine that expresses the SARS CoV-2 spike protein has been developed by AstraZeneca with the University of Oxford. After some initial criticism on their phase I efforts, this vaccine is in large phase II and III trials in England, Brazil, and South Africa.
In China, the company SinoVac, utilizing lessons from prior attempts to develop a SARS CoV vaccine, has developed an adenovirus vector vaccine called CoronaVac that is currently in phase III trials. Another Chinese adenovirus vector vaccine by CanSino Biologics was approved by the Chinese military in late June for limited distribution after only phase II trials - an unprecedented step.
A vaccine developed in Russia, a combination of replication defective adenoviruses, has been announced as leading the vaccine race. However, a paucity of published scientific papers on the vaccine, reflective of a veil of secrecy characteristic of Russian scientific development, has created skepticism pending more convincing publications.
The large number of vaccine candidates, many still in early phases of study, reflects the enormous international effort underway to thwart the seemingly unstoppable spread of COVID-19. Yet, much research remains necessary to determine if vaccination can provide immunity, how long it will last, and whether or when re-vaccination might be necessary.
PARALLELS TO THE 1918 SPANISH INFLUENZA PANDEMIC
It appears that the epilog for the COVID-19 pandemic is still far in the distance. Indeed, in many ways we seem to be reliving the 1918 influenza pandemic. The H1N1 Spanish flu pandemic (which actually was first identified in a Kansas army base) occurred in three massive waves during 1918-19, and killed at least 50 million across the globe, including over 675,000 Americans. It has been estimated that one third of the global population was infected.
There are many parallels. The planet was faced with a devastating, highly contagious, respiratory virus for which there were no therapeutics and no vaccine. The country was gripped by fear that the health care system would totally collapse. Hospitals attempted to increase surge capacity, but were overwhelmed. Social distancing (then called crowd control), face masks, disinfectants, and hand washing were the only weapons.
It was an interplay of biologic and socioeconomic realities. When the biology is fixed, behavior must change. The Wilson White House initially downplayed the severity and extent of disease. The absence of a robust and effective Federal response at that time was, of course, influenced by World War I. There was an active military service draft. Industries were nationalized. The Federal Government left cities and states on their own.
In Philadelphia, the most severely affected city in the United States, a parade was allowed to take place in September 1918, resulting in a massive spike in influenza cases and over 12,000 deaths, which overwhelmed city morgues. Public health interventions were inconsistently applied across the nation. There were mandatory masking orders in San Francisco and Seattle, but they were inconsistent elsewhere. New York City never closed its schools. Distrust and nationalism arose. Blame was levied on foreign countries.
As it was then, so it is now. As Yogi Berra famously said, “It’s like déjà vu all over again.” We can only hope that sane minds and a science-based, rational, and unified approach will soon arrive on the scene.
REFERENCES
1. The Johns Hopkins Coronavirus Resource Center. https://coronavirus.jhu.edu/map.html. Accessed July 27, 2020.
2. Waradon S, Huang N, Becavin C, et al. SARS-CoV-2 entry factors are highly expressed in nasal epithelial cells together with innate immune genes. Nature Medicine 23 April 2020. 26; 681-87
3. Hou YJ, Okuda K, Edwards CE, et al. SARS-CoV-2 Reverse genetics reveals a variable infection gradient in the respiratory tract. Cell May 26, 2020. DOI:https://doi.org/10.1016/j.cell.2020.05.042
4. Tang X, Wu C, Li X, et al. On the origin and continuing evolution of SARS-CoV-2. National Science Review. 2020
5. Korber B, Fischer WM, Gnanakaran S, et al. Tracking changes in SARS-CoV-2 spike: evidence that D614G increases infectivity of the COVID-19 virus. Cell July 2, 2020. DOI:https://doi.org/10.1016/j.cell.2020.06.043
6. Zou L, Ruan F, Huang M, et al. SARS-CoV-2 viral load in upper respiratory specimens of infected patients. N Engl J Med 2020; 382:1177-1179. doi: 10.1056/NEJMc2001737
7. Stadnytskyi V, Bax CE, Bax A, et.al. The airborne lifetime of small speech droplets and their potential importance in SARS-CoV-2 transmission. Proc Natl Acad Sci U S A. 2020; 117(22):11875. Epub 2020 May 13.
8. Ong SWX, Tan YK, Chia PY, et al. Air, surface environmental and personal protective equipment contamination by severe Acute Respiratory Syndrome Coronavirus 2 (SARS CoV-2) from a symptomatic patient. JAMA 2020; 323(16): 1610-1612. Doi: 10.1001/jama.2020.3227.
9. Van Doremalen N, Morris DH, Holbrook MG, et al. Letter. N Engl J Med 2020; 382:1564-1567. DOI: 10.1056/NEJMc2004973
10. Morawska L, and Milton DK. It is time to address airborne transmission of COVID-19. Clin Infec Dis July 6 2020. Accepted manuscript. https://doi.org/10.1093/cid/ciaa939
11. World Health Organization Scientific Brief. July 9, 2020. Transmission of SARS CoV-2: implications for infection prevention precautions. https://www.who.int/news-room/commentaries/detail/transmission-of-sars-cov-2-implications-for-infection-prevention-precautions
12. Chia PY, Coleman KK, Tan YK, et al; Singapore 2019 novel coronavirus outbreak research team. Detection of air and surface contamination by SARS-CoV-2 in hospital rooms of infected patients. Nat Common. 2020; 11(1):2800. doi:10.1038/s41467-020-16670-2
13. Wong SCY, Kong RT, Wu TC, et al. Risk of nosocomial transmission of coronavirus disease 2019: an experience in a general ward setting in Hong Kong. J Hosp Infect. 2020; 105(2):119-127. Epub 2020 Apr 4.
14. Oran DP, Topol EJ. Prevalence of asymptomatic SARS-CoV-2 Infection: A narrative review. Ann Intern Med 2020. June 3; M20-3012. doi: 10.7326/M20-3012.
15. He X, Lau EHY, Wu P, et al. Temporal dynamics in viral shedding and transmissibility of COVID-19. Nat Med. 2020; 26(5):672-675. doi:10.1038/s41591-020-0869-5
16. Widders A, Broom A, and Broom J. SARS-CoV-2: The viral shedding vs. infectivity dilemma. Infect Dis Health. 2020 May 20:S2468-0451(20)30028-6. doi: 10.1016/j.idh.2020.05.002.
17. Walsh KA, Jordan K, Clyne B, et al. SARS CoV-2 Detection, Viral load, and infectivity over the course of an infection: J. Infect. 2020 Jun 29; S0163-4453(20)30449-7. doi: 10.1016/j.jinf.2020.06.067
18. Bullard J, Dust K, Funk D, et al. Predicting infectious SARS-CoV-2 from diagnostic samples. Clin Infect Dis. 2020; May 22. doi: 10.1093/cid/ciaa638
19. Cheng HY, Jian SW, Liu DP, et al. Contact tracing assessment of COVID-19 transmission dynamics in Taiwan and risk at different exposure periods before and after symptom onset. JAMA Intern Med. 2020; May 1;e202020. doi: 10.1001/jamainternmed.2020.2020
20. Lui M, Cheng SZ, Xu KW, et al. Use of personal protective equipment against coronavirus disease 2019 by healthcare professionals in Wuhan, China: cross sectional study. BMJ 2020; 369:m2195. doi: https://doi.org/10.1136/bmj.m2195
21. Steensels D, Orix E, Coninx L, et al. Hospital-wide SARS-CoV-2 antibody screening in 3056 staff in a tertiary center in Belgium. JAMA 2020 Jun 15; [e-pub]. https://doi.org/10.1001/jama.2020.11160
22. Rivett L, Sridhar S, Sparkes D, et al. Screening of healthcare workers for SARS-CoV-2 highlights the role of asymptomatic carriage in COVID-19 transmission. eLife 2020; 9:e58728. https://doi.org/10.7554/eLife.58728
23. Chu DK, Aki EA, Duda S, et al. Physical distancing, face masks, and eye protection to prevent person-to-person transmission of SARS-CoV-2 and COVID-19: a systematic review and meta-analysis. Lancet 2020; 395(10242): 1973-1987. doi: https://doi.org/10.1016/S0140-6736(20)31142-9
24. Liu Z, Bing X, Zhi XZ. The epidemiological characteristics of an outbreak of 2019 novel coronavirus diseases (COVID-19)—China, 2020. China CDC Weekly. 2020 Feb 10;41(2):145-151. doi:10.3760/cma.j.issn.0254-6450.2020.02.003.
25. Polak SB, Van Gool IC, Cohen D, et al. A systematic review of pathological findings in COVID-19: a pathophysiological timeline and possible mechanisms of disease progression. Modern Path June 22, 2020. https://doi.org/10.1038/s41379-020-0603-3.
26. Xu Z, Shi L, Wang Y, et al. Pathological findings of COVID-19 associated with acute respiratory distress syndrome. Lancet Respir Med. 2020; 8(4):420-422. doi:10.1016/S2213-2600(20)30076-X
27. Klok FA, Kruip MJHA, van der Meer NJM, et al. Incidence of thrombotic complications in critically ill ICU patients with COVID-19. Thromb Res. 2020; 191:145-147. doi:10.1016/j.thromres.2020.04.013
28. Zhou F, Yu T, Du R, et al. Clinical course and risk factors for mortality of adult inpatients with COVID-19 in Wuhan, China: a retrospective cohort study. Lancet. 2020; 395:1054–1062.
29. Aboubaker A, Raba AA, and Aboubaker A. Extrapulmonary and atypical clinical presentations of COVID-19. J Med Virology June 10, 2020. https://doi.org/10.1002/jmv.26157
30. Behzad S, Aghaghazvini L, Radmard AR, et al. Extrapulmonary manifestations of COVID-19: Radiologic and clinical overview. Clin Imaging 2020 Oct; 66: 35-41. doi: 10.1016/j.clinimag.2020.05.013
31. Dufort EM et al. Multisystem inflammatory syndrome in children in New York State. N Engl J Med 2020 Jun 29; [e-pub]. doi: https://doi.org/10.1056/NEJMoa2021756.
32. Sedaghat Z, and Karimi N. Guillain Barre Syndrome associated with COVID-19 infection: a case report. J Clin Neurosci 2020 Jun; 76: 233-235. doi: 10.1016/j.jocn.2020.04.062
33. The Severe Covid-19 GWAS Group. Genome-wide association study of severe Covid-19 with respiratory failure. N Engl J Med 2020 Jun 17; [e-pub]. doi: https://doi.org/10.1056/NEJMoa2020283.
34. Beigel JH, Tomashek KM, Dodd LE, et al. Remdesivir for the treatment of COVID-19: preliminary report. N Engl J Med. published online May 22, 2020. doi:10.1056/NEJMoa2007764.
35. Li L, Zhang W, Hu Y, et al. Effect of convalescent plasma therapy on time to clinical improvement in patients with severe and life-threatening COVID-19: a randomized clinical trial. JAMA. Published online June 3, 2020. doi:10.1001/jama.2020.10044
36. The RECOVERY Collaborative Group. Dexamethasone in hospitalized patients with COVID-19: preliminary report. N Eng J Med. Published online July 17, 2020. https://www.nejm.org/doi/10.1056/NEJMoa2021436
37. Alzghari SK, Acuña VS. Supportive treatment with tocilizumab for COVID-19: a systematic review. J Clin Virol. 2020; 127:104380. doi:10.1016/j.jcv.2020.104380
38. Guaraldi G, Meschiari M, Cozzi-Leori A, et al. Tocilizumab in patients with severe COVID-19: a retrospective cohort study. Lancet Rheum June 24, 2020. doi: doi:https://doi.org/10.1016/S2665-9913(20)30173-9
39. Centers for Disease Control and Prevention. June 30, 2020. Interim clinical guidance for management of patients with confirmed coronavirus disease (COVID-19) https://www.cdc.gov/coronavirus/2019-ncov/hcp/clinical-guidance-management-patients.html
40. Wang W, Xu Y, GAO R, et al. Detection of SARS-CoV-2 in different types of clinical specimens. JAMA. 2020; 323(18):1843-1844. doi:10.1001/jama.2020.3786
41.Guo L, Ren L, Yang S, et al. Profiling early humoral response to diagnose novel coronavirus disease (COVID-19). Clin Infect Dis. published online March 21, 2020. doi:10.1093/cid/ciaa310
42. Long Q-X, Tang XJ, Shi QL, et al. Clinical and immunological assessment of asymptomatic SARS-CoV-2 infections. Nat Med 2020 Jun 18; [e-pub]. doi: https://doi.org/10.1038/s41591-020-0965-6.
43. Sekine T, Perez-Potti A, Rivera-Ballesteros O, et al. Robust T cell immunity in convalescent individuals with asymptomatic or mild COVID-19. BioRxIV. June 2020; 1-25. doi: https://doi.org/10.1101/2020.06.29.174888
44. Lurie N, Saville M, Hatchett R, et al. Developing Covid-19 vaccines at pandemic speed. N Engl J Med. 2020; 382(21):1969-1973. doi:10.1056/NEJMp2005630