Spring 2018 - Vol. 13, No. 1
Back to the Future, or Folly Redux?
Joseph M. Kontra, M.D.
Lancaster General Health Physicians Infectious Diseases
Great fleas have little fleas upon their backs to bite ’em
And little fleas have lesser fleas, and so on ad infinitum…
—Augustus de Morgan (1806-1871)
ABSTRACT
In an age of worsening antibiotic resistance and a dwindling supply of new antibiotics, scientists are beginning to rediscover an antibacterial treatment that pre-dated the discovery of antibiotics, and which may hold promise of a “new” therapeutic modality for specialists in Infectious Diseases. This paper provides an overview of this exciting area of active research.
INTRODUCTION
Bacteriophages, or phages for short, are viruses that can infect and kill bacterial cells. They are believed to be the most abundant life form on earth, consisting of at least 100 million different species
1 with an estimated biosphere burden of 10
32 phage particles in existence at any given moment.
2 This viral predation of bacteria is responsible for the estimated killing of half of the bacteria on this planet every 48 hours.
3 Such antagonistic competition over a billion years of evolution has played a major role in the incredible genetic diversity and high mutation rates of both predator and prey.
4
Bacteriophages are ubiquitous. These self-replicating nano-particles are found in large numbers in water (especially sewage), soil, and throughout the GI tract and skin surface of virtually all living creatures.
STRUCTURE AND LIFE CYCLE OF PHAGES
Phages contain a double-stranded DNA core surrounded by a capsule of lipoprotein. They typically have a protein tail used for surface receptor recognition and cell wall binding, as well as a contractile sheath that facilitates a syringe-like injection of viral DNA into the host cell (Fig. 1). Phages are highly specific in their tropism, often infecting only specific strains of a bacterial species. As such, phages can be utilized in strain identification schemes in research laboratories.
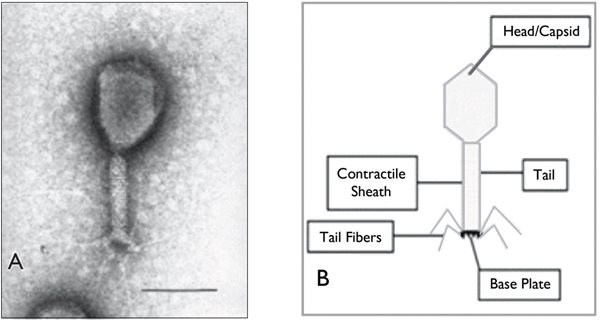
Fig. 1. Appearance of a typical phage, with a protein tail used for surface receptor recognition and cell wall binding, and a contractile sheath that facilitates a syringe-like injection of viral DNA into the host cell. (From: Deresinski S. Bacteriophage Therapy: Exploiting Smaller Fleas. Clin Infect Dis 2009. 48(8):1096)
When phage DNA enters a parasitized bacterial cell, host RNA polymerase transcribes the DNA, which results in one of two life cycles (Fig. 2). One pathway after infection of the bacterial cell can lead to replication and assembly of new virions (complete viral particles), resulting in the destruction of the bacterial cell by lysis and release of progeny phages. It is this lytic cycle that makes phages of interest as potential direct therapeutic agents against bacteria pathogenic to humans.
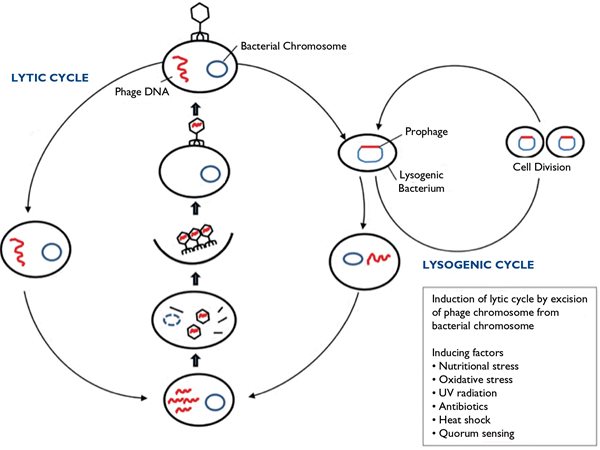
Fig. 2. Diagram of the two possible life cycles after phage DNA enters a parasitized bacterial cell and host RNA polymerase transcribes the DNA. (From: Knoll et al. Antibacterial Bioagents Based on Principles of Bacteriophage Biology: An Overview. Clin Infect Dis. 2014;58(4):528)
In the alternative pathway, the delivered phage DNA may become incorporated into the host genome, replicate with the bacterial cell, and remain silent in a lysogenic cycle that can be responsible for transduction, i.e. the transmission of genetic elements between bacteria. Since this transmission of DNA can include antibiotic resistance factors, it may provide an opportunity for antibacterial genetic engineering, as we will see later.
A BRIEF HISTORY OF BACTERIOPHAGE SCIENCE
Viruses of bacteria were first discovered in 1915 by Frederick Twort,
5 who observed that cell-free supernatants were able to kill bacterial cell cultures. By 1917 the field was expanded by d’Herelle,
6 and was fueled by the already recognized therapeutic potential of these bacterial viruses in both animals and humans.
Early experimentation with human phage therapy was carried out in France and Belgium, where children with dysentery or cholera showed an apparent clinical response when treated with the filtrates of stool from recovered soldiers. Similarly, there seemed to be anecdotal success after treatment of Staphylococcus furuncles with a local injection of phage suspension, and of
Staphylococcus aureus bacteremia with an IV infusion of suspended phage particles.
7
The potential and pitfalls of early quasi-scientific phage treatment in the pre-antibiotic era are exemplified by this sobering 1936 description
8 of a dying patient treated with intravenous phage therapy for
Staphylococcus aureus bacteremia:
“The temperature is taken every 2 hours to record the lysis. When it occurs it is massive and accompanied by shock. The temperature falls within 3-5 hours to 37-40 degrees and below. From the time of the decrease in temperature the patient feels truly reborn; his face brightens, and the observer sees a true resurrection. The theoretical objections to the intravenous treatment with phage and the possibility of introducing un-lysed filtered pathogens do not hold up against the fact of definitive cure.”
Despite such anecdotal reports, the enthusiasm of the 1920s and 1930s was ultimately dampened by a lack of fundamental understanding of these viruses, limited availability of technology, and poorly reproducible experimental results. Several studies in the English language literature, including a review in
JAMA by Krueger et. al
9 questioned the scientific validity of the entire field. After the discovery of antibiotics, as well as the devastation of WWII, research in phage therapy fell out of favor.
Bacteriophage therapy remained dormant, yet still viable to some extent in France, Eastern Europe, and particularly in the Republic of Georgia and the Soviet Union. Quasi-scientific and anecdotal publications claiming efficacy in a broad array of bacterial illness were met with skepticism from the West, where phage research was almost non-existent.
Bacteriophage therapy was rediscovered in the English language literature in the 1980s, with more scientifically sound reports of successful phage therapy in animals with E. coli gastrointestinal infections.
10 Research accelerated in the 1990s with advances in understanding the fundamental biology of bacterial viruses, as well as the dawn of bacterial and viral genomics.
NON-HUMAN COMMERCIAL UTILIZATION OF BACTERIOPHAGES IN THE UNITED STATES
To combat enteric bacterial contamination in foods, Intralytix Inc has produced three FDA-approved products for topical application. These include a 6-phage cocktail called ListShield that can be sprayed on cheese and meats to prevent infection with
Listeria monocytogenes,
11 an organism that replicates more rapidly at refrigeration temperatures. Additional products include EcoShield, a phage cocktail active against
E. coli 0157:H7, and SalmoFresh, active against
Salmonella enteritica strains. A product designed to treat ready-to-eat foods with a 5-phage cocktail of Shigella-specific bacteriophages is in development, and has received GRAS (Generally Recognized As Safe) status from the FDA.
12
Other areas of research into potential commercial applications of phage treatments include agriculture, veterinary medicine, and waste water management. Recall that sewage contains perhaps the highest concentration of naturally occurring bacteriophages.
POTENTIAL HUMAN APPLICATIONS OF PHAGE THERAPY
Knowledge about the biology of phages has steadily increased over the last three decades. The properties of rapid bacterial killing, target specificity, and continued replication at the target site, give phage therapy intriguing potential for treating bacterial infections in humans. This potential has been enhanced by studies of multiple routes of administration – topical, oral, inhalation, injection, and infusion – and by advances in genetic engineering.
13
Lysis of bacteria can release endotoxin and other detrimental biochemical compounds. Genomic manipulation has, for example, produced lysis-deficient phages that can kill the target bacteria without release of inflammatory substances.
14
Phages can also potentially be utilized as therapeutic delivery systems for antibacterial molecules. Examples include intracellular delivery of toxins as well as genetic induction of antibacterial protein synthesis by infected bacteria.
15 Another area of research is genomic manipulation through phage-mediated genetic engineering of the bacterial target cell.
In orthopedic and other implant infections, bacteria produce a biofilm that provides an environment that protects against host immunity, and prevents penetration of antibiotics. In an experimental model of MRSA infection of orthopedic implants, phage treatment inhibited biofilm formation and facilitated antibiotic efficacy, resulting in greater MRSA eradication rates than with either modality alone.
16
In cholera epidemics, fluctuations in the seasonal prevalence of the disease are inversely correlated with the naturally fluctuating prevalence of environmental V. cholera phages.
17 Experimental protocols for phage treatment of cholera-contaminated water are currently being developed.
SUPPORTIVE EXPERIMENTAL STUDIES IN ANIMALS
For many patients with chronic lung disease, persistent colonization by Gram-negative bacteria, with recurrent infections and the progressive development of antibiotic resistance, is a reality. The feasibility of phage therapeutics has been studied in experimental models of infections in chronic lung disease.
Patients with cystic fibrosis are susceptible to complex and potentially lethal lung infections with multi-drug-resistant (MDR)
Burkholderia cepacia. In a mouse model, the administration of aerosolized phage particles significantly reduced the lung tissue bacterial burden, thus facilitating immunologic bacterial clearance.
18
In a murine model of pulmonary infection with
Burkholderia cenocepacia (one of the multiple species within the
Burkholderia cepacia complex), systemic administration of phage agents proved superior to inhalation administration in reducing infection, without generating a detrimental host inflammatory response.
19
In a mouse model of pulmonary infection with MDR
Pseudomonas aeruginosa, specific phage therapy not only reduced bacterial burden, but also reduced biofilm production.
20
In a gastrointestinal infection model, phage treatment afforded protection of mice against a lethal inoculum of highly virulent MDR
Vibrio parahaemolyticus, demonstrating its use both as a potential bio-control agent as well as a therapeutic intervention.
21
One issue with the above studies is that the phage agents were administered very soon after the infecting bacteria were introduced. We still lack experimental models of more established and pathologically advanced infections.
PHAGE SYNERGY WITH ANTIBIOTICS
In an experimental rat model of
Pseudomonas aeruginosa endocarditis, a 12-phage cocktail was compared to antimicrobial therapy alone, and to a combination of phage infusion and antibiotics. The combination group proved superior to either phage or antibiotic therapy alone in sterilizing vegetations. Further, phage particles easily penetrated the vegetations, and were demonstrated to continue to replicate within them.
22
PHAGE LYSINS AS THERAPEUTIC MODALITIES
The use of lysins, the chemical products of phages, has been proposed as a therapeutic method to kill bacteria that takes advantage of bacteriophages’ potential while steering clear of their complex biology. External application of phage-derived lysins has been studied as a treatment for Gram-positive organisms such as Anthrax, Group-A Streptococcus, and
Streptococcus pneumoniae.
23 In an experimental model of pneumococcal septicemia, for example, intravenous infusion of a phage lysin resulted in eradication of pneumococci from the blood.
24 Unfortunately, targeting of Gram-negative bacteria proves to be more problematic due to a more complex outer cell membrane.
A
Clostridium difficile bacteriophage has been developed and sequenced, and its endolysin has been harvested. This chemical product has been shown to be active against a wide variety of C. difficile isolates, including ribotype 027/B1/NAP1.
25
PHAGE THERAPY IN HUMANS
Phage therapy continues to be used in Eastern Europe and Russia. While there are no large-scale randomized controlled trials of phage therapy in humans in the West, phase I and II trials have begun to appear.
In phase I trials, phage and topical antibiotic combined therapy of chronic leg ulcers caused by Pseudomonas,
S. aureus, and
E. coli has been well tolerated, although only marginally efficacious.
26 In a phase I trial of patients with drug-resistant Pseudomonas otitis, phage therapy has been well tolerated and has resulted in a significant decrease in bacterial burden.
27
An exception to the narrow strain spectrum of bacteriophages may be
Staphylococcus aureus, where a single phage can result in lysis of over 700 strains.
28 Research is underway on the treatment of
S. aureus rhino-sinusitis, as well as the potential use of phages for decolonization of the nares, which is critical to efforts to reduce surgical site infections.
A study of phage therapy for gastrointestinal infections due to
E. coli in children showed no benefit over standard oral rehydration therapy. However, interpretation of this study’s results has been complicated by mixed pathogen isolation and an unclear establishment of the cause of the diarrhea.
29
In addition, there are recent anecdotal reports of clinical success with intravenous phage therapy. A critically ill patient with an infected pancreatic pseudocyst and a progressive downhill ICU course over several months due to disseminated infection with a highly resistant
Acinetobacter baumannii was treated with experimental phage cocktails under an Emergency Investigational New Drug application with the FDA. The organism was tested against phage libraries from the University of Texas A&M Center for Phage Technology, the Naval Medical Research Center, and the biotech firm AmliPhi Biosciences, Inc. After endotoxin removal and other painstaking purification steps, multiple phage cocktails were administered first by intra-peritoneal injection, and then – after a sub-optimal response – by intravenous administration. The patient began to improve significantly within 48 hours of the initiation of intravenous phage therapy. Phage therapy continued for 8 weeks, during which time the patient gradually recovered and was discharged on hospital day 245.
30
While an N of 1 does not a revolution make, the scientific rigor of this anecdotal report has yielded a number of informative microbiologic findings germane to the science of potential phage therapy. First, successive patient isolates of Acinetobacter developed resistance to the initial phage cocktail within eight days, demonstrating that phage therapy can be a moving target. Secondly, even though subsequent Acinetobacter isolates from the patient were resistant to the initial phage cocktail, the bacteria demonstrated a shift in antimicrobial resistance, with increased sensitivity to minocycline, as well as a loss of its bacterial capsule. In addition, phage therapy seemed to mitigate against the rapid development of resistance to minocycline that would have been expected in the setting of single drug therapy. And lastly, synergistic killing of the Acinetobacter islolate by the combination of phage and minocycline was demonstrated in vitro.
BARRIERS TO THE FURTHER DEVELOPMENT OF HUMAN PHAGE THERAPY
Despite the accumulating evidence listed above, progress toward widespread application of phage therapy for routine human use remains problematic. FDA guidelines remain to be developed for this burgeoning field of study. Phages are, after all, living organisms, not drugs, thus adding layers of complexity to processes for regulation, development of clinical trials, and approval of therapeutic modalities.
Keep in mind that bacteriophages have high mutation rates and strain specificity. Clinical utilization would require identifying not only the genus and species of the pathogenic bacteria in a particular patient, but the bacteria’s strain type and phage susceptibility pattern as well. An entire library of phages readily accessible to the clinician would be required. In addition, effective phage therapy in experimental models requires the use of phage cocktails, often mixing many phage types together to minimize the development of resistance and to maximize the effect. Cold chain stability and mutational drift are additional concerns for these delicate microbes if we are to harness their potential. And, of course, the target specificity of bacteriophages would preclude their use as an empiric treatment.
As generations of phages evolve through lysis, genetic material from the bacterial genome can be incorporated into the phage genome. Thus, there is concern that detrimental Trojan horse antibiotic resistance gene segments could be accidentally transmitted to the target bacterial strain.
Phages are highly variable in their in vivo behavior. Can the science of pharmacology accurately describe the behavior of live phages in humans? Imagine if an antibiotic could self-replicate within the human host. How would that change the pharmacology of the drug? Such wide variation in phage “pharmacokinetics” and “pharmacodynamics” within the human host will certainly slow the deployment of phage therapy until this new science develops.
Finally, while anti-phage antibodies during treatment do not appear to be detrimental to the mammalian host, the full spectrum of consequences to the human immune system remains to be elucidated.
PHAGE THERAPY AND BIG PHARMA
There are currently at least a half dozen pharmaceutical firms actively developing phage therapies for a wide variety of applications. The cost of research and development compared to chemical agents, however, may prove staggering. As we have noted, phages exhibit different physical, biological, and in vivo behaviors compared with antibiotics. Understanding and controlling the complex biology of phages will itself be a rate-limiting step in the process. In addition, current federal approval processes will need to be either extensively modified, or new processes created. The target specificity of phages that makes them so attractive clinically will limit the broad utilization/sale of phage agents. All of this will further add to the cost of phage research and development to the point that such development may not be economically viable from a business perspective.
31
CONCLUSION
While there seems to be tremendous potential for the development of human phage therapeutics, the obstacles seem equally daunting. Clearly human phage therapy will never obviate the need for continued development of newer, broader, and more potent antibiotics. Its niche will be limited to persistent infections with Multi-Drug Resistant (MDR) organisms, both by the extreme bacterial strain specificity of phages, and the current need to test phage libraries extensively in the laboratory to derive appropriate phage cocktails. Nonetheless, given the current accelerating pace of antimicrobial resistance, human phage therapy may evolve to be an important addition to our armamentarium, and thus is a goal we must pursue.
REFERENCES
1. Rohwer F. Global phage diversity. Cell 2003;113:141.
2. Hendrix R. Bacteriophages: evolution of the majority. Theor Popul Biol 2002;61:471.
3. Pal C, et al. Coevolution with viruses drives the evolution of bacterial mutation rates. Nature 2007;450:1079
4. Deresinski S. Bacteriophage Therapy: Exploiting Smaller Fleas. Clin Infect Dis 2009. 48(8):1096
5. Twort FW. An investigation on the nature of ultramicroscopic viruses. Lancet 1915; 186:1241-3
6. d’Herelle F. About an invisible microbe antagonistic to dysentery bacilli. Comte Rend Acad Sci 1917;165:372
7. Abedon ST, et al. Phage Treatment of human infections. Bacteriophage 2011;1(2):66
8. Suave L. Le bacteriophage in chirurgie. La Medecine 1936. 17:49
9. Krueger AP, Scribner EJ. The bacteriophage. Its nature and its therapeutic use. J Am Med Assoc 1941; 116:2160-7.
10. Smith HW. The control of experimental E. coli diarrhea in calves by means of bacteriophages. J Gen Microbiol 1987; 133:111
11. Intralytix. Available at:
http://www.intralytix.com/Intral_Human.htm.
12. Whitworth JJ. Intralytix phage-based Shigella tech backed by FDA.
https://www.foodqualitynews.com/Article/2017/04/18/GRAS-backing-for-Intralytix-Shigella-intervention
13. Pires DP, et al. Genetically engineered phages: a review of advances over the last decade. Microbiol. Mol. Biol. Rev. 2016;80:523
14. Matsuda T, et al. Lusos-deficient bacteriophage therapy decreases endotoxin and inflammatory mediator release and improves survival in a murine peritonitis model. Surgery 2005;137:639.
15. Westwater C. et al. Use of genetically engineered phage to deliver antimicrobial agents to bacteria: an alternative therapy for treatment of bacterial infections. Antimicrob. Agents. Chemother. 2003;47:1301
16. Yilmaz C, et al. Bacteriophage therapy in implant-related infections: and experimental study. J Bone Joint Surg. Am. 2013;95:117.
17. Faruque SM, et al. Seasonal epidemics of cholera inversely correlate with the prevalence of environmental cholera phages. Proc Natl Acad Sci USA 2005;102:1702.
18. Semler DD. Aerosol phage therapy efficacy in Burkholderia cepacia complex respiratory infections. Antimicrob Agents and Chemother 2014. 58(7);4005
19. Carmody LA, et al. Efficacy of bacteriophage therapy in a model of Burkholderia cenocepacia pulmonary infection. J. Infect. Dis. 2010;201(2);264.
20. Waters EM, et al. Phage therapy is highly effective against chronic lung infections with pseudomonas aeruginosa. Thorax.2017;72(7):666.
21. Jun JW, et al. Bacteriophage Therapy of a Vibrio parahaemolyticus infection caused by a multiple-antibiotic-resistant O3:K6 pandemic clinical strain. J. Infect Dis. 2014;210(1);72.
22. Oechslin F. Synergistic interaction between phage therapy and antibiotics clears pseudomonas aeruginosa infection in endocarditis and reduces virulence. J. Infect Dis 2016;215(5):703.
23. Fischetti V. Bacteriophage lysins as effective antibacterials. Curr Opin Microbiol 2008;11:393.
24. Loeffler J, et al. Phage lytic enzyme Cpl-1 as a novel antimicrobial for peuoccal bacteremia. Infect Immun 2003;71:6199.
25. Mayer M. Molecular characterization of a Clostridium difficile bacteriophage and its cloned biologically active endolysin. J. Bacteriol 2008;1190:6734.
26. Rhodes DD, et al. Bacteriophage therapy of venous leg ulcers in humans: results of a phase I safety trial. J. Wound Care 2009;18:237. Doi:10.12968/jowc.2009.18.6.42801
27. Wright A, et al. A controlled clinical trial of a therapeutic bacteriophage preparation in chronic otitis due to antibiotic-resistant Pseudomonas aeruginosa; a preliminary report of efficacy. Clin Otolaryngol 2009;34:349. Doi:10.1111/j.1749-4486.2009.01973.x
28. Pantucek R, et al. The polyvalent staphylococcal phage 0812; its host range mutants and related phages. Virology 1998;246:241.
29. Sarker SA. From bench to bed and back again: phage therapy of childhood Escherichia coli diarrhea. Ann NY Acad Sci 2016;1372:42. Soi:10.1111/nyas.13087.
30. Schooley RT et al. Development and use of personalized bacteriophage-based therapeutic cocktails to treat a patient with disseminated resistant Acinetobacter baumannii infection. Antimicrob. Agents Chemother. 2017. Doi:1128/AAC.00954-17.
31. Cooper CJ, et al. Adapting drug approval pathways for bacteriophage-based therapeutics. Frontiers in Microbiology 2016:7:1209. Doi: 10.3389/fmicb.2016.01209.